Combining cutting-edge materials for more efficient, sensitive gaseous sensors
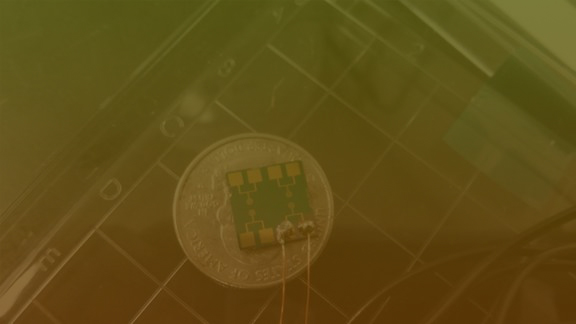
The human nose can distinguish among a trillion different combinations of smells. Even so, there are plenty of gases that our noses can't detect at the level of sensitivity we need. That's where gaseous sensors come in. While some of the first sensors were animals – like canaries in coal mines – we've since replaced them with technologies that can detect miniscule amounts of chemicals in the air.
Just like our own noses, gaseous sensors are essential for safety and comfort. In factories, gaseous sensors can alert managers to chemical leaks or processes running incorrectly. Outside, they measure pollutants, helping cities monitor air quality. In homes, they keep family members safe. Building managers use measurements from humidity and temperature sensors to maximize energy efficiency.
These sensors wouldn't exist without a fundamental understanding of chemistry and physics. This basic knowledge helps scientists understand how and why sensing materials interact with gaseous chemicals. Many cutting-edge materials have promise for use in sensors, if only scientists can learn how to better produce and control them.
"Sensors are where materials research meets environmental detection," said Pete Beckman, a researcher at Department of Energy's Argonne National Laboratory (ANL).
To set the groundwork for innovation, the DOE Office of Science funds projects and user facilities that support sensors research.
Creating the Materials for Sensing
Like noses, sensors rely on a combination of components to detect and make sense of gases or chemicals in the air. In humans, molecules float up into your nose and bind to special neurons. Neurons then pass the message up to the brain. In sensors, the material inside the sensor acts as a neuron. When that material interacts with a chemical in the air, it may emit light, change its ability to conduct electricity, or shift shape. The materials and electronics around the sensing material communicate that message to the sensor's "brain," whether that brain is a computer or a warning signal like a siren.
Developing sensors' nervous systems and brains is a job for applied science. Fundamental research like the work at the Office of Science's laboratories sets the foundation for that applied science. In particular, this research is expanding scientists' understanding of the materials themselves and how to produce them.
Three types of cutting-edge materials offer huge potential for use in sensors: nanoparticles, two-dimensional (2-D) materials, and metal-organic frameworks (MOFs). Nanoparticles are miniscule particles that are bigger than atoms, but act fundamentally differently from larger particles of the same substance. 2-D materials, like graphene, form sheets only a single atom thick. MOFs are compounds made of metal ions linked together by carbon-based connectors.
All of these materials have humongous surface areas compared to their overall sizes. Because lots of gas molecules can interact with their surfaces, they can be sensitive to tiny amounts of chemicals. In addition, scientists can craft all of these materials into a variety of structures. That customization could allow researchers to create specialty materials to detect a particular chemical.
Zinc Sulfide Nanoparticles
The key to building a better sensor may lie in making its sensing material out of nanoparticles. Unfortunately, it's challenging to manufacture some of the most promising of those nanoparticles. Sensors for hydrogen and other gases already use the material zinc sulfide. Producing zinc sulfide in nanoparticle form could make it cheaper and more effective. But the current process for producing zinc sulfide nanoparticles involves very high temperatures, pressures, and toxic chemicals.
Scientists at DOE's Oak Ridge National Laboratory (ORNL) investigated a cheaper, more efficient nanoparticle production process. Researchers supported by both DOE's Advanced Manufacturing Office and Office of Science found that microbes may offer an alternative path forward.
Not just any bacteria will do. Scientists used Thermoanaerobacter, a bacteria that normally lives in extremely hot places with no oxygen. After adding a cheap sugar and chemicals that included zinc and sulfur, the bacteria produced about three-quarters of a pound of zinc sulfide nanoparticles. The process was 90 percent cheaper than current methods.
Growing 2-D Materials
Two-dimensional materials are a special form of nanomaterial that are only a few atoms thick. They have so much surface area compared to their volume that they provide a lot of space for gas molecules to interact and are able to hold a large number of them. But 2-D materials act so differently from their normal "bulk" counterparts that scientists don't have a good grasp on how they grow. Without this understanding, manufacturers can't consistently produce high-quality versions of them.
To tackle this problem, ORNL scientists explored a better way to grow 2-D material gallium selenide (GaSe). As they grew the material in a container filled with argon gas, they found that by changing the temperature and flow of the gas, they could switch back and forth between laying down and taking away atoms. But just discovering how to change back and forth between the two states didn't tell them what was actually happening on the chemical level.
"To visualize what we were doing in the lab, we needed high-resolution, state-of-the-art facilities as well as in-situ diagnosis tools," said Tolga Aytug, an ORNL scientist. To get that level of precision, the team turned to the Center for Nanophase Materials Sciences, an Office of Science user facility at ORNL. The tools there helped them see how the processes they used to grow the material affected its structure and properties. Based on that information, they refined their methods to get the characteristics they wanted.
In the future, scientists may be able to combine various 2-D materials into thin, versatile sensors. "The beauty of 2-D materials is that you can stack the different layers together to make some artificial material," said Kai Xiao, an ORNL scientist. These artificial materials would be able to detect a variety of different chemicals instead of just a single one.
Metal-Organic Frameworks
The metal ions and carbon-based connectors of MOFs form open, cage-like structures. A MOF only a few inches wide has an amazing 2.5 acres of surface area. That provides plenty of space for molecules to interact with.
As a result, MOFs can sense minute levels of chemicals. Scientists control which chemicals they want a MOF to detect by changing the size of its spaces, its shape, or how its parts link to each other.
"For a MOF-based sensor to work, it has to be very selective and very sensitive," said Praveen Thallapally, a scientist at DOE's Pacific Northwest National Laboratory (PNNL).
One benefit specific to MOFs is their ability to accommodate new molecules by changing their structures. PNNL scientists found a MOF with a zinc base could capture cobalt and copper. When these metals exited the molecule, the MOF returned to its original structure. This means after a chemical attaches to a MOF and triggers a sensor, someone could reset and reuse the sensor without needing to replace the MOF.
Much of the ongoing research into MOFs focuses on how to discover and build them. MOFs' traditional starting materials are rigid and difficult to work with. In contrast, polymers (flexible chains of molecules) are easier to control. However, they usually bunch together in dense, disorganized clumps. To draw on the advantages of each, scientists from the University of California, San Diego found a way to use polymers to build MOFs. Using both allows researchers to combine MOFs' consistency and large surface area with polymers' ease of use. The researchers used the hybrid materials to create thin films, which are typically used in sensors.
The next breakthrough in MOF research may come from computer modeling. Using trial and error to figure out which structure will interact best with a specific chemical could take years and be very expensive. In contrast, powerful computer models using machine learning allow scientists to find just the right material in a few days.
PNNL scientists searching for a MOF that could select between xenon and krypton collaborated with the National Energy Research Scientific Computing Center, an Office of Science user facility at DOE's Lawrence Berkeley National Laboratory. After searching through more than 120,000 options, their computer model pointed to a calcium-based material that excelled at this task.
Connecting the Nose to the Body
A great sensing material is essential, but it won't work by itself. Just as a nose needs a body and brain, sensing materials need to be part of a bigger mechanism. Unfortunately, getting these materials to work together within a sensor is often a challenge.
Printing Nanoparticle Ink
"Ink" made of sensing nanoparticles printed onto paper, plastic, rubber, or fabric could allow engineers to create smaller and more flexible sensors.
"Making particles is one thing. But from those particles, making a functional ink is not trivial," said ORNL scientist Pooran Joshi, in a slight understatement.
One ORNL study tackled the best way to turn copper-based nanoparticles into high-quality ink. By shining a high-intensity light for only a few millionths of a second, scientists fused the nanoparticles together without melting the surface underneath. When the copper-based nanoparticle ink fused together, it created a printed surface. Researchers then used the printed surface as a component in a temperature sensor.
Combining Nanotubes and Nanocrystals
Scientists know that sensors made of nanotubes and nanocrystals could detect as little as one part per million of a gas – if only they can get these two materials to work together.
Ralu Divan and her team at ANL discovered a way to add nanocrystals of zinc oxide – which is already used in sensors – to carbon nanotubes. Sensors that use the two together could be far more sensitive to methane than current technology. By placing the zinc oxide nanocrystals down atom by atom, they created a thin, consistent layer on top of the nanotubes. With this process, companies can precisely control the zinc oxide's thickness and coverage.
To examine the bonds between the nanocrystals and nanotubes, the team relied on the Center for Nanoscale Materials, an Office of Science user facility at ANL. "Having everything in one place has saved a lot of time and we were able to move faster than we expected," said Divan.
As a result, they developed a sensor that could detect much lower concentrations of methane than previous ones. Operators can use it again in seconds instead of minutes or hours.
This sensor improved so much on the existing technology that in 2016, R&D 100 Magazine recognized it as an R&D 100 finalist. The research team is now working with the Array of Things project, a collaboration between the University of Chicago and ANL. As part of the effort to collect real-time data from hundreds of sensors across Chicago, the Array of Things team anticipates using these methane sensors in the future.
Projects such as the Array of Things have the potential to transform cities into networks of sensors, placing digital eyes and noses throughout the built landscape. But these networks and technologies wouldn't be possible without a solid scientific foundation. Nothing may match the human nose's versatility, but research the Office of Science is supporting helps fill in the gaps of our biological capabilities.